New challenges need new technologies to tackle them. Here, the World Economic Forum identifies the top most promising technology trends that can help to deliver sustainable growth in decades to come as global population and material demands on the environment continue to grow rapidly. These are technologies that the Council considers have made development breakthroughs and are nearing large-scale deployment.
OnLine Electric Vehicles (OLEV)
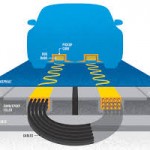
On-Line Electric Vehicle(OLEV) can be wirelessly charged on the road. OLEV has pick-up devices built in under the vehicle to collect the magnetic field generated by power cables under the road and convert the energy into electricity for EV operation. Hence, no extra time is required for recharging the battery as the OLEV can get the electric power while driving on the power line. This new idea makes OLEV distinct from Plug-in Electric Vehicle(PEV). OLEV uses the road as a charging station, so there is no need to build costly charging facilities and it can reduce the battery size dramatically.
The core technology of OLEV is Shaped Magnetic Field in Resonance (SMFIR). This technology enables the delivery of a large amount of energy to an electric vehicle safely, while it is stationary or in motion. With this technology, power is transferred from the ground to the bottom of the vehicle, 20cm apart, while maintaining power transmission efficiency at 80%. Also, with up to 100kW of power supply, the technology brings OLEV much closer to its commercial applications. This technology meets all the safety standards of electromagnetic waves.
3-D printing and remote manufacturing
3-D Printing is a process of making a three-dimensional solid object of virtually any shape from a digital model. 3D printing is achieved using an additive process, where successive layers of material are laid down in different shapes. 3D printing is also considered distinct from traditional machining techniques, which mostly rely on the removal of material by methods such as cutting or drilling .
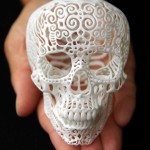
A materials printer usually performs 3D printing using digital technology. The first working 3D printer was created in 1984 by Chuck Hull of 3D Systems Corp. Since the start of the 21st century there has been a large growth in the sales of these machines, and their price has dropped substantially. According to Wohlers Associates, a consultancy, the market for 3D printers and services was worth $2.2 billion worldwide in 2012, up 29% from 2011.
The 3D printing technology is used for both prototyping and distributed manufacturing with applications in architecture, engineering, construction (AEC), industrial design, automotive, aerospace, military, engineering, civil engineering, dental and medical industries, biotech (human tissue replacement), fashion, footwear, jewelry, eyewear, education, geographic information systems, food, and many other fields. It has been speculated that 3D printing may become a mass market item because open source 3D printing can easily offset their capital costs by enabling consumers to avoid costs associated with purchasing common household objects.
Self-healing materials
Self-healing materials are a class of smart materials that have the structurally incorporated
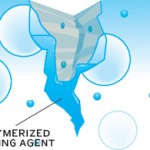
ability to repair damage caused by mechanical usage over time. The inspiration comes from biological systems, which have the ability to heal after being wounded. Initiation of cracks and other types of damage on a microscopic level has been shown to change thermal, electrical, and acoustical properties, and eventually lead to whole scale failure of the material. Usually, cracks are mended by hand, which is unsatisfactory because cracks are often hard to detect. A material (polymers, ceramics, etc.) that can intrinsically correct damage caused by normal usage could lower production costs of a number of different industrial processes through longer part lifetime, reduction of inefficiency over time caused by degradation, as well as prevent costs incurred by material failure. For a material to be defined strictly as self-healing, it is necessary that the healing process occurs without human intervention.
Carbon dioxide (CO2) conversion and use
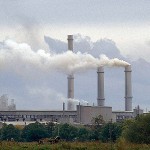
The need to cut CO2 emission into the atmosphere is pushing scientists and technologists to discover and implement new strategies that may be effective for controlling the CO2 atmospheric level (and its possible effects on climate change). One option is the capture of CO2 from power plant flue gases or other industrial processes to avoid it entering the atmosphere. The captured CO2 can be either disposed in natural fields (geological cavities, spent gas or oil wells, coal beads, aquifers; even oceans have been proposed) or used as a source of carbon in synthetic processes. In this paper, we present the options for CO2 utilization and make an analysis of possible solutions for the conversion of large volumes of CO2 by either combining it with H2, that must be generated from water, or by directly converting it into fuels by electrolysis in water using solar energy. A CO2–H2-based economy may address the issue of reducing the environmental burden of energy production, also saving fossil carbon for future generations. The integration of CO2 capture and utilization with CO2 capture and storage would result in a more economically and energetically viable practice of CO2 capture.
Remote sensing
Remote sensing is the acquisition of information about an object or phenomenon without making physical contact with the object. In modern usage, the term generally refers to the use of aerial sensor technologies to detect and classify objects on Earth (both on the surface, and in the atmosphere and oceans) by means of propagated signals (e.g. electromagnetic radiation emitted from aircraft or satellites).
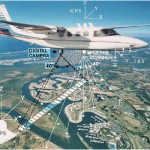
There are two main types of remote sensings: passive remote sensing and active remote sensing Passive sensors detect natural radiation that is emitted or reflected by the object or surrounding areas. Reflected sunlight is the most common source of radiation measured by passive sensors. Examples of passive remote sensors include film photography, infrared,charge-coupled devices, and radiometers. Active collection, on the other hand, emits energy in order to scan objects and areas whereupon a sensor then detects and measures the radiation that is reflected or backscattered from the
target. RADAR and LiDAR are examples of active remote sensing where the time delay between emission and return is measured, establishing the location, speed and direction of an object. Remote sensing makes it possible to collect data on dangerous or inaccessible areas. Remote sensing applications include monitoring deforestation in areas such as the Amazon Basin, glacial features in Arctic and Antarctic regions, and depth sounding of coastal and ocean depths. Military collection during the Cold War made use of stand-off collection of data about dangerous border areas. Remote sensing also replaces costly and slow data collection on the ground, ensuring in the process that areas or objects are not disturbed. Orbital platforms collect and transmit data from different parts of the electromagnetic spectrum, which in conjunction with larger scale aerial or ground-based sensing and analysis, provides researchers with enough information to monitor trends such as El Niño and other natural long and short term phenomena. Other uses include different areas of the earth sciences such as natural resource management, agricultural fields such as land usage and conservation, and national security and overhead, ground-based and stand-off collection on border areas.
Precise drug delivery through nanoscale engineering
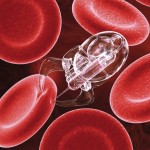
In pharmaceutical industries, a new molecular entity (NME) that demonstrates potent biological activity but poor water solubility, or a very short circulating half-life, will likely face significant development challenges or be deemed undevelopable. On the other hand, less active but pharmaceutically optimal compounds may become more suitable candidates for development. In either case, there is always a degree of compromise and such trade-offs may inevitably result in the production of less-ideal drugs. However, with the emerging trends and recent advances in nanotechnology, it has become increasingly possible to address some of the shortcomings associated with potential NMEs. By using nanoscale delivery vehicles, the pharmacological properties (e.g. solubility and circulating half-life) of such NMEs can be drastically improved—essentially leading to the discovery of optimally safe and effective drug candidates. This is just one example that demonstrates the degree to which nanotechnology may revolutionize the rules and possibilities of drug discovery and change the landscape of pharmaceutical industries. Indeed, current nanotechnology-based therapeutic products have been validated through the improvement of previously approved drugs, and many novel classes of nanotherapeutics are now underway1-3.
Drug discovery is only one of the many areas in healthcare that nanotechnology is now benefiting. The current and promising applications of nanomedicine include, but are not limited to, drug delivery, in vitro diagnostics, in vivo imaging, therapy techniques, biomaterials, and tissue engineering. Summarized in Box 1 are some important opportunities that nanotechnology may afford in each research area. Some of these opportunities are becoming realities or are actually being used today, while others are generating promise in their early phases of development and are expected to experience vigorous growth in the foreseeable future. As recognition of the importance of this exciting field, it is expected that the global market of nanoscale applications in the medical field could grow to $70 – $160 billion by 2015.4,5 In this perspective.
Organic electronics and photovoltaics
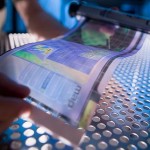
Organic electronics and photovoltaic technology are reaching critical mass with the establishment of a U.S. consortium and the recent introduction of new products into the marketplace. Manufacturing of products based on organic semiconductors, however, continues to be hindered by poor reproducibility and process-dependent properties. We develop an integrated suite of measurement methods to tie the electrical and photovoltaic performance of organic semiconductor devices to the materials structure of the layers produced by the manufacturing processes. By providing the measurement link for the structure-processing-performance paradigm, our methods will rationally accelerate product development, enable standard measurements and increase manufacturing output through science-based process design and process control approaches.
Developing commercial products based on organic electronics requires materials that deliver predictable and reproducible performance. One advantage of these materials is their compatibility with versatile solution processing methods. However, this advantage can lead to unpredictable performance and poor reproducibility because the critical microstructure of the material forms dynamically as the solution dries during manufacturing. Many process parameters influence the drying process and the microstructure formation is often hard to control.
A combination of spectroscopic tools (infrared, visible and X-ray), diffraction and scanning probe microscopy provides sufficiently detailed characterization to isolate the contributions of individual structure and processing variables. This integrated measurement platform provides a rational basis for informing process design, and it will further accelerate materials development by separating the molecular basis for electric performance from the process-induced variability. In situ variations on fast and low-cost versions of these techniques will provide a robust platform for science-based process control.
Fourth-generation reactors and nuclear-waste recycling
Researchers aim to produce safe nuclear fuel that can be 80 percent recycled, compared to the current 1 percent; these fourth generation nuclear power systems can lead to a reduction of the amount of high-level, long-lived nuclear waste to a tenth of what it is today, while energy output can increase hundredfold With a €9.4 million budget, a group of European researchers are collaborating to investigate nuclear fuel manufacturing and recycling for the fourth generation nuclear power systems. The aim is to produce safe fuel that can be 80 percent recycled, compared to the current 1 percent. Chalmers University of Technology in Gothenburg, Sweden, is in charge of the initiative.
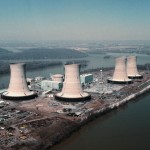
A Chalmers University of Technology release reports that fourth generation nuclear power systems can lead to a reduction of the amount of high-level, long-lived nuclear waste to a tenth of what it is today, while energy output can increase hundredfold. Many researchers believe the new technology will have a commercial breakthrough within twenty years. Germany is at present the only European country that has decided to phase out nuclear power.
“The technology needed for the fourth generation already exists,” says Christian Ekberg, professor and nuclear chemistry research team leader at Chalmers. “What is needed now is for the different parts to be connected. One important aspect involves integrating nuclear waste recycling into the cycle so that nuclear power plants can be built with facilities to recycle waste and produce new nuclear fuel on site.”
Christian Ekberg is also the inaugural holder of Stena Metall’s professorship in Industrial Materials Recycling, and is the coordinator of the new research project called Asgard , which has received €5.5 million in EU grants.
Around fifty European researchers will take part in the project over four years.
“Traditionally, three different groups have worked separately on the fourth generation nuclear power systems: reactor physicists, fuel chemists and separation chemists. The groups will cooperate within the Asgard project to tie their previous findings together. We will also perform research on entirely new reactor fuels that are safer, use resources more effectively and that enable a more comprehensive approach to the waste issue.”
Oxides currently dominate among the nuclear fuels that are produced from recycled nuclear waste. One example is MOX fuel. During the course of the Asgard project, researchers will examine other types of chemical compounds with uranium or plutonium. Examples include nitrides and carbides. These chemical compounds are safer to use in reactors, amongst other things because their high melting point and thermal conductivity offer a higher safety margin in terms of a nuclear meltdown.